Energies RenouvelablesMines Géologie
Mineral Demand Surge: Effects on Energy Transition Resilience
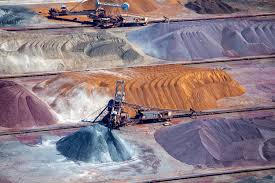
The demand for minerals is projected to rise significantly by 2030 in alignment with the emission reduction targets of the Paris Agreement. This increase is driven by the needs of electricity networks, battery storage, electric vehicles, and renewable energies, all essential for a comprehensive energy transition (Figure 1) [1].
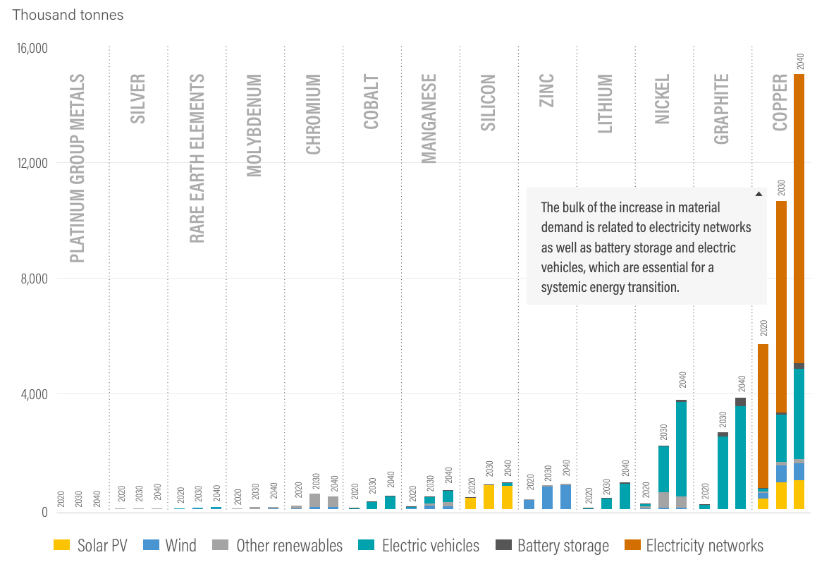
Figure 1: Anticipated growth in material demand by technology according to the International Energy Agency (IEA) Sustainable Development Scenario for 2030 and 2040 compared to 2020. Source: REN21 [1].
Renewable energy technologies rely on various minerals, many classified as critical (Table 1). The demand for these materials varies by technology, with wind turbines, solar PV modules, and energy storage systems requiring a diverse array of resources. Copper is prevalent across all renewable technologies, while rare earth elements like dysprosium, neodymium, praseodymium, and terbium are increasingly in demand, particularly for wind turbines. Cobalt, graphite, and lithium are essential for battery storage [1].
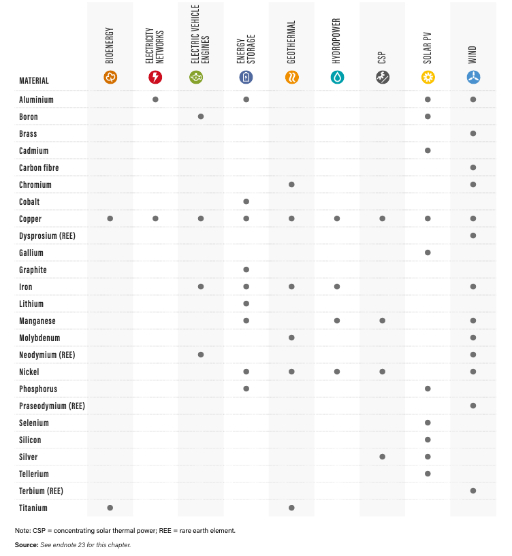
Table 1: Materials used in various renewable energy technologies. Source: REN21 [1].
Current supply from existing mines and projects under development is expected to meet only half of the projected demand for lithium and cobalt and 80% of the demand for copper by 2030. The criticality of materials for renewable energy manufacturing arises not only from the supply-demand gap but also from long development timelines for mining projects, geographical concentration of production, declining resource quality, and associated environmental and social challenges [2].
The geographical concentration of materials for the renewable energy transition is greater than that of oil and natural gas [1, 2]. The top three producing countries control about 75% of global supply for cobalt, lithium, and rare earth elements. China and the Democratic Republic of the Congo account for 70% of global cobalt production and 60% of 1rare earth elements. Australia leads in lithium supply, while South Africa dominates platinum production, each holding over half of their markets. This concentration extends to processing, with China controlling over 50% of processing for cobalt, lithium, and rare earth elements, and more than 30% for copper and nickel. Such concentration makes supply chains vulnerable to disruptions from natural disasters, political instability, or regulatory changes, as seen with recent export restrictions on lithium from Zimbabwe, nickel ore from Indonesia, and rare earth elements from China [1].
Global solar photovoltaics (PV) capacity has increased five-fold from 2015 to 2022 and is expected to grow by 300-400% annually over the next two decades. Solar PV systems consist of modules, inverters, trackers, mounting structures, and various electrical components, with material intensities varying by module type. The predominant technology, crystalline silicon (c-Si), holds a 95% market share in 2023. Other technologies include cadmium telluride (CdTe), copper indium gallium diselenide (CIGS), and amorphous silicon (a-Si).
A standard c-Si module typically contains aluminum for the frame, glass for the casing, 5% silicon for solar cells, 1% copper, and less than 1% silver for interconnectors.
Thin-film technologies such as CdTe, CIGS, and a-Si require fewer minerals than c-Si, but need more glass. CdTe modules use cadmium and tellurium, while CIGS modules rely on gallium and selenium, eliminating the need for silver or silicon. Innovations in manufacturing and design have significantly reduced material usage; for instance, in 2021, c-Si modules designs showed a 50% reduction in silicon needed per watt of capacity and an 80% reduction in silver compared to 2008 [1].
In 2022, global concentrated solar power (CSP) capacity reached approximately 6.3 GW. Despite a slowdown in growth, projections indicate capacity could increase forty-fold by 2040 [1]. CSP technologies use mirrors or lenses to concentrate sunlight, converting it into high-temperature heat to power traditional steam turbines or engines for electricity generation [3]. These technologies also serve various industrial processes requiring low (below 150°C) to medium (150-400°C) process heat, such as water desalination, food processing, and chemical and mineral processing [1]. The most common CSP technologies for electricity generation are parabolic troughs and central towers. While parabolic troughs have historically dominated the market, central towers offer higher efficiency and greater storage capacity, positioning them to lead the CSP sector in the future [1, 3].
Materials for CSP towers typically include steel and concrete, with mirrors (heliostats) reflecting sunlight and steam turbine equipment made from stainless steel containing 9-12% chromium, as well as molybdenum, manganese, nickel, vanadium, and carbon. Parabolic troughs consist of multiple parabolic mirrors arranged in parallel rows, tracking the sun from east to west daily, ensuring that solar energy is continuously focused on the receiver pipes [3].
Common reflective materials include specialized solar-grade glass mirrors coated with aluminum. Silver and aluminum are the most effective coating materials, providing optimal reflectance in the reflecting layers of CSP
concentrators.
Copper is utilized for wiring, pumps, electric motors, and generators. In central towers, molten salts serve both as a heat storage medium and for heat transfer, whereas parabolic trough systems use synthetic oil that flows through stainless steel tubes.
Approximately 8 billion tonnes of coal, 4 billion tonnes of oil, and the equivalent of 2.6 billion tonnes of fossil gas are extracted annually from the Earth’s land and sea floors (Figure 2).
In 2022, the combustion of these fossil fuels released about 35 gigatonnes of CO2 equivalent into the atmosphere [1].
In addition to being a significant contributor to global climate change [4], the extraction and combustion of fossil fuels cause air, water, and soil pollution, which have extensive effects on ecosystems and human health [5, 6].
The critical material requirements for fossil fuels include various resources essential for their extraction, processing, and distribution (Figure 2). For coal, key materials such as steel and cement are vital for constructing mining equipment and infrastructure, alongside transport systems like rail lines and shipping ports. In the oil sector, steel is extensively used in drilling rigs, pipelines, and storage tanks, while composite materials enhance the strength and reduce the weight of advanced drilling equipment. Natural gas extraction relies on similar materials, requiring steel and alloys for high-pressure pipelines and specialized infrastructure for liquefaction and regasification. Additionally, common materials across fossil fuels include copper for electrical systems and plastics for insulation and piping [1].
Nickel is used in the refining of crude oil, particularly in hydroprocessing, where it serves as a catalyst to remove impurities from fuels. Cobalt plays a crucial role in lithium-ion batteries, enhancing their stability and performance, and is also used in fuel cell technologies that convert chemical energy from fossil fuels directly into electricity.
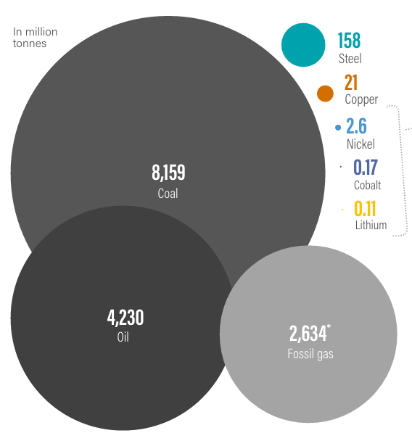
Figure 2 : Yearly production of selected energy-related fuels and minerals. Source: REN21 [1].
In 2021, nuclear energy contributed 10% to global electricity production and accounted for 4% of total primary energy consumption in 2022. Nuclear power plants utilize enriched uranium as fuel and incorporate steel and concrete for reactor and containment structures, along with boron as a neutron absorber. The fuel assemblies within the reactor are typically housed in zirconium alloy tubes, while some plants use graphite as a moderator to slow down neutrons and enhance the fission process. Additional materials required for these plants include copper, aluminum, stainless steel, insulation, and wiring. In 2021, approximately 62,496 tonnes of uranium were needed to fuel operational nuclear power plants worldwide. According to the International Atomic Energy Agency, around 8 million tonnes of conventional uranium resources remained in the ground as of early 2019. Plutonium, produced as a by-product in the reactor, contributes to over one-third of the energy generated by nuclear power plants [1].
Most non-renewable energy sources—such as fossil fuels and nuclear power—do not depend on materials considered
« critical, » except for copper and aluminum, which are primarily required for grid connectivity.
Overall, this article serves as a comprehensive resource for understanding the complexities of mineral demand in the
context of the global energy transition. Its insights can inform policymakers, researchers, and industry stakeholders
about the critical role of minerals in achieving sustainability goals.
References:
[1] REN21. (2023). Renewable Energy and Sustainability Report. REN21 Secretariat, Paris, France. Available at:
https://www.ren21.net/wp-content/uploads/2019/05/REN21-RESR-2023_LowRes.pdf
[2] Bouramdane, A.-A. (2023). « Minéraux de la Transition Énergétique : Criticité Géologique, Géostratégique et Environnementale. »
Énergie/Mines & Carrières. DOI: 10.5281/zenodo.7594617. Available at: https://energiemines.ma/mineraux-de-la-transition-energetique-criticite-geologique-geostrategique-et-environnementale/
[3] Bouramdane, A-A. (2021), « Scenarios of Large-Scale Solar Integration with Wind in Morocco: Impact of Storage, Cost, Spatio-Temporal Complementarity and Climate Change ». Institut Polytechnique de Paris, [PhD Thesis], Physics, Oct 2021, https://tel.archives-ouvertes.fr/tel-03518906
[4] Bouramdane, A.-A (2022). Assessment of CMIP6 Multi-Model Projections Worldwide: Which Regions Are Getting Warmer and Are Going through a Drought in Africa and Morocco? What Changes from CMIP5 to CMIP6? Sustainability, 15, 690. https://doi.org/10.3390/su15010690
[5] Bouramdane, A.-A. (2024), « Morocco’s Path to a Climate-Resilient Energy Transition: Identifying Emission Drivers, Proposing Solutions, and Addressing Barriers », EDP Sciences – Science and Technology for Energy Transition (STET), Formerly « Oil & Gas Science and Technology (OGST) » – supported by « IFPEN (IFP Energies nouvelles) » and « CEA (French Alternative Energies and Atomic Energy Commission) », https://doi.org/10.2516/stet/2024021
[6] Bouramdane, A-A. (2024), « Impacts du Changement Climatique sur la Santé Publique et Solutions pour des Villes Durables », 91e Congrès de l’Acfas | Colloque 641 – Villes-Santé et Développement Durable, 14-15 mai 2024, Université d’Ottawa, UIR-Center for Global Studies, École Polytechnique Fédérale de Lausanne (EPFL),
https://www.acfas.ca/evenements/congres/programme/91/600/641/c
By Ayat-Allah Bouramdane, Assistant Professor – Researcher at the International University of Rabat (IUR).
